Introduction
This article introduces the various backhaul technologies available to 5G networks and focuses on E-band wireless radio links and how they will enable the continued deployment of 5G networks globally. It will provide a technical analysis of the necessary system requirements for E-band technology. We then map the results into the physical radio design, while offering insights into the millimeter wave (mmW) signal chain.
Topology of a 5G Network
5G networks are deploying in greater numbers, based on the success of 4G long-term evolution (LTE) technology. Figure 1 outlines the topology of a 5G Network to help visualize the radio network from access to backhaul. The topology outlines four scenarios with a separate connection back to the core network.
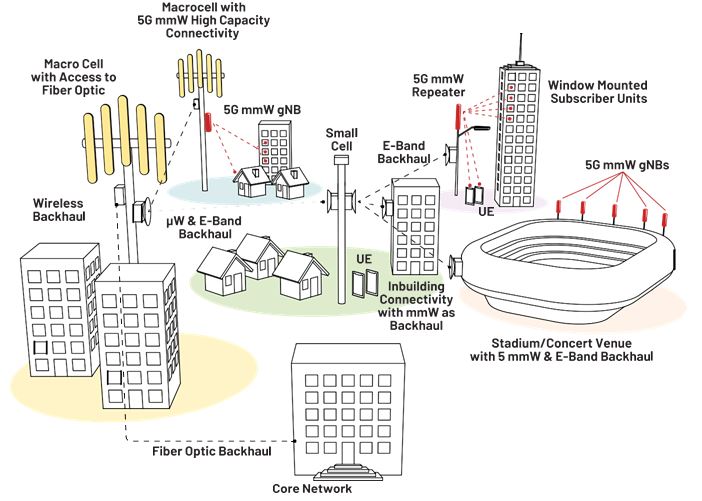
User equipment (UE) such as a cell phone and 5G wireless internet will access the network by connecting to the base station (gNodeB) in the next-generation radio access network (NG-RAN). In Figure 1, we represent gNodeB as the macrocells, small cells, 5G mmW access points, and repeaters. Macro and small cells cover the frequency range (FR) from 410 MHz to 7.125 GHz (FR1). 5G mmW solutions cover the frequency range from 24.25 GHz to 52.6 GHz (FR2). Macrocells have a large coverage radius, while small cells, which are higher in number than macrocells, are easier to deploy but have a smaller radius of coverage. Their function is to handle traffic in dense areas and add capacity or coverage to the network in a more efficient way without adding more macrocells. 5G mmW is the latest generational technology that adds capacity to support new user experiences that increase network capacity demand such as a live sporting event where fans could watch replays on a mobile device. There are several further instantiations of NG-RAN equipment operating in FR1 and FR2 such as massive MiMO radios, microcells, femtocells, picocells, etc.
The term backhaul, or mobile backhaul, refers to the transport network that connects the core network (CN) and the radio access network (gNodeB in 5G). With increased cell site densification, the importance of mobile and fixed wireless backhaul is evident as high capacity links to the core network are needed. The 2022 Ericsson Microwave Outlook report shows that 5 Gbps to 20 Gbps per site backhaul capacity will be required for urban cell sites by 2025. In Figure 1, we show wireless backhaul as both microwave (μW) and E-band (mmW) radios. E-band radios can be colocated with μW radios or as a higher data bandwidth alternative to μW radios. While new business opportunities are enabled using 5G, there is a growing pressure on mobile operators to quickly deliver (time-to-market) high capacity, low latency, reliable, scalable, and cost-optimized backhaul links in urban or rural locations.
What Is the Difference Between Backhaul, Midhaul, and Fronthaul?
In 5G RAN, the baseband unit (BBU) functionality is split into both distributed unit (DU) and centralized unit (CU). How the operator chooses to locate them is up to available fronthaul interface and link transport technology and how much processing is best done at the edge in a low latency manner vs. a more centralized one. Figure 2 shows the architectural evolution of the radio access network. Backhaul is a core part of each solution.
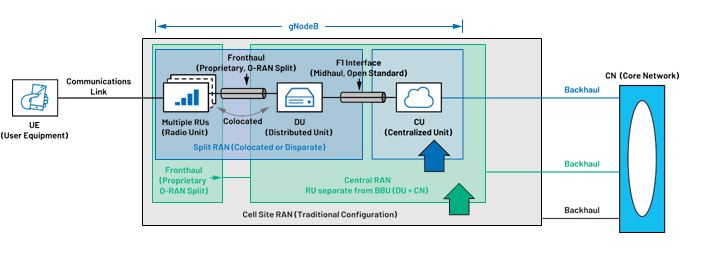
- Cell site RAN: Traditional configuration where the radio unit (RU) and BBU functionality is housed at a cell site. A separate backhaul link connects to the core network.
- Centralized RAN (low level split): This model allows for portions of the network to be centralized to the edge site and in doing so delivers virtualization benefits (vBBU). This pushes processing capability to the edge site and reduces the complexity at the cell site as only the physical layer is present there. However, a fronthaul link is now necessary to transport large amounts of data between the RU and centralized BBU. This is sometimes referred to as a low level split.
- Split RAN (high level split): This allows for the RU and DU to be colocated at the cell site or they can be located separately. It promises to deliver virtualization benefits (vBBU) along with cost efficiencies. The CN is located separately at the edge site. This is referred to as a high level split:
- RU and DU colocated at cell site, while CN is located at the edge site. This means a midhaul link is required to connect a remote CN (edge site) to the RU + DU (cell site).
- RU, DU, and CN located separately.
Both centralized and split RAN models allow for multiple vendor hardware and software implementations, which should bring cost efficiencies to the network deployment. The equipment must be interoperable (RU, DU, CU) to allow mixing and matching between vendor solutions to capture the efficiencies. This is at the heart of the open RAN (O-RAN) alliance. Traditionally, this has not been the case as equipment providers had proprietary interface solutions that prohibited interoperability with multiple vendor’s equipment.
What is interesting is fronthaul and midhaul are now links that are evolving as operators deploy in centralized and split RAN configurations. E-band can provide an excellent solution where fiber is not available and/or the installation of such would be cost-prohibitive or not a viable near-term deployable solution.
It’s worth noting that a core difference between 4G and 5G is that in 5G NR the traditional EPC (evolved packet core), which runs on specialist hardware and is usually located at the base or near the cell tower, was split up. This allows individual functions to run on commercial off-the-shelf (COTS) hardware. So, in effect, the core network in 5G is more decentralized as functions have moved to the edge. See Figure 3. Core network functions can now be colocated at the edge making communications faster and decreasing latency for users. This also allows for network slicing that creates virtual networks for specific application requirements. For example, a slice could provide high speed broadband while another slice might provide machine-to-machine connectivity for IoT. Also, this edge cloud architecture enables edge computing. So, networks can have small data centers close to the edge to support, for instance, video streaming of the same content rather than trying to backhaul the data from a centralized location. In general, this 5G architecture creates efficiencies and more flexibility in how to architect the network access, hardware, functions, and backhaul.
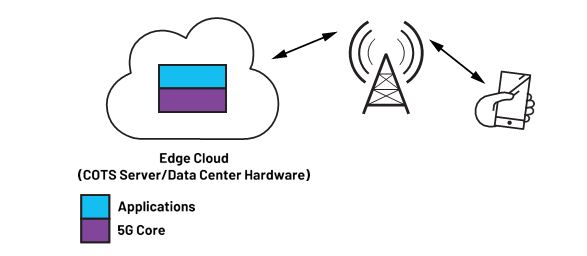
What Are the Available Backhaul Solutions Today?
Fiber backhaul is the highest capacity option available to mobile network operators (MNOs). This is the dominant small cell backhaul technology in use today due to the availability of fiber in many densely populated urban/indoor areas where small cells are used for coverage/capacity increases. Fiber has a capacity up to 1.6 Tbps (160 signals × 10 Gbps per signal). Fiber is the highest capacity choice for MNOs. However, the main challenges with fiber deployment are cost, availability, planning permission (logistics), and ultimately time to deploy. According to GMSA, the cost to deploy fiber is approximately $70k/km. CAPEX and the time to deployment are always barriers to continued growth. It must be noted that μW/mmW backhaul and fiber are complementary solutions and coexist in networks. Wireless and fiber offer the operators alternate backhaul technologies. The optimal backhaul solution has many factors to consider including, time to deployment, federal, state, and city permitting, obtaining right of way access, data bandwidth requirements, terrain, and total cost of ownership.
μW and mmW backhaul is the dominant backhaul technology for macrocells to date and accounts for approximately 50% of macrocell backhaul links.
μW licensed band technology is very capable, easy to deploy, and relatively low cost (there’s no need to dig up city streets or lay trenches). It covers frequencies from 6 GHz to 42 GHz and those bands are very suitable for medium to long range links covering up to 25 km.
mmW backhaul within V band (57 GHz to 66 GHz) and E-band (76 GHz/86 GHz) has existed for several years. V band however suffers from significant oxygen absorption that creates a large signal attenuation at 60 GHz. Also, countries have different regulations when it comes to this band’s use. Some, license portions of the spectrum for backhaul, while others have left it for unlicensed use. Europe and U.S. are territories that allow license-exempt use and are implementing rules to reduce the probability of interference for different configurations, but still, V band is unreliable when it comes to high quality backhaul. Its use is instead forecast primarily for unlicensed indoor and outdoor coverage solutions at short range (WiGig). E-band offers a broader bandwidth, lower impairment solution that will deliver high availability links.
So why hasn’t E-band featured more in networks in the past? In 4G networks mmW backhaul technology was underutilized given the available bandwidth capacity, which was only needed in certain scenarios, so most of the wireless backhaul was done using the licensed μW bands (6 GHz to 42 GHz). This is changing with the explosive rollout of 5G networks and densification, which is demanding 10 Gbps or higher backhaul capability.
So, what are some core advantages of using E-band and how does it stack up against fiber and μW? E-band offers two 5 GHz bands of the spectrum from 71 GHz to 76 GHz and from 81 GHz to 86 GHz. The bands are subdivided into multiple channels of 250 MHz. A key advantage in the spectrum allocation is that it can be used for time division duplex or frequency division duplex links. Capacity is also not an issue, as the maximum amount of data that can be transmitted in a licensed E-band point to point link is greater than 60 Gbps.1 E-band also has the potential to be used in point to multipoint systems, which will further increase the available backhaul data bandwidth. There is significantly more channel capacity compared to the traditional μW radios, which are limited to roughly 2.4 Gbps links due to frequency availability. Also, because E-band antennas concentrate electromagnetic energy in a tightly concentrated beam of energy (for example, 1 degree of directivity), they can be of a high gain (45 dBi), small form factor (30 cm antenna diameter) radio nclosure, ideal for discrete placement on buildings or towers. And with a modest RF transmit power, it’s common for E-band to support link lengths up to 3 km.2 See Table 1 for a comparison among the most popular backhaul technologies.
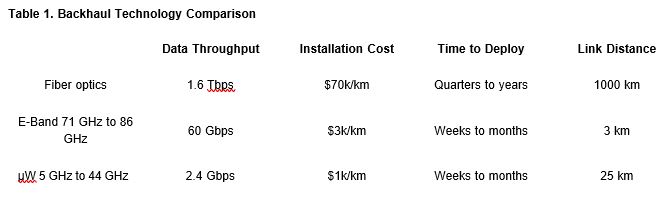
Copper is a legacy technology using T1/E1 protocol. Copper does not scale easily to provide the bandwidth needed for 4G not to mind 5G. It is an option still for indoor small cells and public venues but operators are moving away from this technology. Satellite is not widely used in comparison to fiber or μW/mmW as the data rates are limited and due to geostationary satellites (very high Earth orbit), the latency is problematic. Low Earth orbit (LEO) satellites may have an increasing role to play here with their improved latency, but this is still unclear. The key advantage of satellite is connecting rural areas where alternative means are not possible. Wi-Fi is not a well-used technology for backhaul, except only in a very small number of emerging markets. The frequency bands are unlicensed and thus inference from the growing number of wireless access points and limited range is an issue.
How Does Wireless E-Band Link Transfer Data Over the Air?
E-band uses traditional digital modulation coding such as from BSPK to 1024 QAM. But what can limit the link distance?
- Adverse weather: Rain, fog, sleet, and snow will attenuate the signal strength in an unpredictable manner, decreasing the signal level presented to the receiver, which reduces the signal-to-noise ration (SNR). Interestingly, when presented with rain fade, E-band radio links can use adaptive modulation. This means a link can move to a less complex modulation to prevent data loss. This will maintain the link connectivity for high availability data links by reducing the capacity during this time. The ADI system in package (SiP) solution will provide 99.999% availability for a 1 km link with up to 100 mm/hr of rain.
- Baseband capability: When operating at the E-band frequencies, the baseband unit becomes the bottleneck for data throughput. The typical BBU will support 10 Gbps of data throughput, while the spectrum available can support greater than 60 Gbps of data throughput. The ADI E-band SiPs will support modulation orders up to 1024 QAM.
- Phase noise of LO: Phase noise can limit the modulation order. LO jitter will contribute to lower SNR because of noise that gets superimposed on the signal of interest to be upconverted/downconverted. ADI has excellent wideband external phase-locked loop/voltage-controlled oscillator (PLL/VCO) sources along with E-band on-chip LO path multipliers and amplification.
Table 2 shows the expected bit efficiency and SNR requirements for multiple modulations supported by E- band technologies.
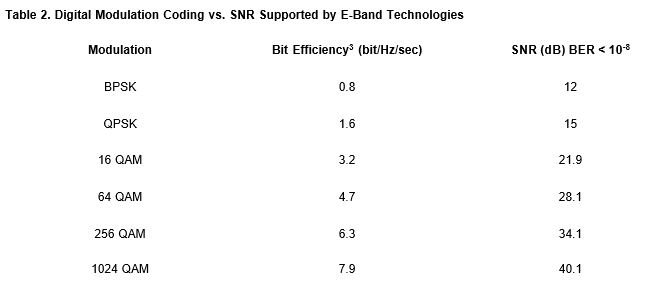
Are E-Band Radios More Di cult to Design than μW radios?
It is surprising to note that E-band radios can leverage much of the current μW radio baseband card design including modem core, processor, memory modules, clock recovery/generation, and sync 1588 circuitry along with the lower frequency analog front end. This provides an easier transition for μW radio vendors to move into the E-band space. See Figure 4 for reference. The E-band front-end module, diplexer, and antenna are those new design blocks, required to transition a μW radio to an E-band radio.
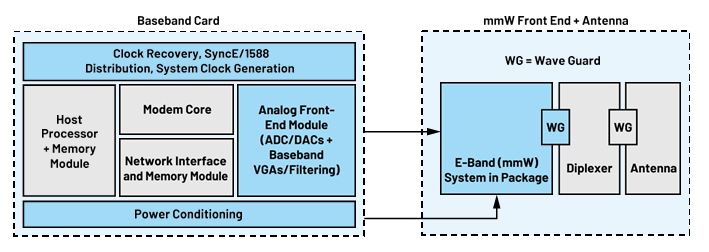
No doubt a 76 GHz/86 GHz design can seem intimidating as there is an increased complexity in mmW design vs. the lower frequency RF or even μW. As noted in Figure 4, wave guide transitions are now integrated as part of the ADI E-band SiP to transition to higher frequency signals with minimal radio frequency (RF) loss as possible to the antenna. The ADI SiPs have eliminated die, bonding, and epoxy assembly. The ADI SiPs can be assembled with standard pick and place assembly equipment. The E-band SiP has made radio assembly similar to μW radio assembly.
E-band link budgets can be challenging due to the free space loss of 131 dB at 1 km4 and rain attenuation of 17 dB/km and 31 dB/km for 99.99% and 99.999% availability. 5 Designers must carefully consider requirements such as gain, transmit power, noise figure, and IP3 to meet the 5G network operator backhaul requirements.
Analog Devices has a rich heritage in μW and mmW backhaul technology. It has developed E-band devices to reduce many of the design and assembly challenges mentioned above to help more designers feel comfortable working in this E-band space.
E-Band—The Next Worthy Contributor to Meet 5G Backhaul Demands
This exploration of E-band highlights its increased bandwidth for 5G networks expanding backhaul options. It is an excellent complementary technology to fiber and gives operators even more flexibility as they plan their deployments and balance a centralized to split RAN solution.
ADI removed much of the heavy lifting associated with E-band front-end design by developing surface mount, highly integrated SiPs with baseband input or output and an integrated waveguide output or input. Designers no longer must worry about handling die and instead can utilize ADI’s E-band packaged technology solutions. At ADI, our goal is to enable this market but offering more accessible technology to the wider RF/μW and mmW design community. Part 2 will delve into an E-band link budget, and the technical details of the ADI E- band SiP family of products.
References
Remmert, Harrald. “What Is 5G Network Architecture?” Digi International, Inc., 2021. “Mobile Backhaul: An Overview.” GSM Association, June 2019.
“Ericsson Microwave Outlook Report: October 2022.” Ericsson, 2022.
Authors
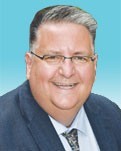
Andy Boyce
Andy Boyce is a system architect at Analog Devices developing signal chains and system solutions. His career spans over 30 years designing RF and microwave products for cable, wireless, and defense systems. Andy holds a B.S.E.E degree from University of Massachusetts Lowell and an M.S.F. degree from Bentley University.
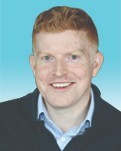
Donal McCarthy
Donal McCarthy is the marketing and business development director for the Microwave Communications Group at Analog Devices in Cork, Ireland. Donal holds a B.E.E. degree from the University College Cork, an M.B.A. degree from Boston College, and a marketing degree from Irish Management Institute in Dublin. Donal held various roles including design engineer at MACOM, field sales engineer and marketing roles at Hittite, and marketing manager and director roles at ADI.